L7 Ion channels and transporters
一、Ion Channels
Patch Clamp Method 膜片钳
The first direct evidence for the presence of voltage-sensitive, ion-selective channels in nerve cell membranes came from measurements of the ionic currents flowing through individual ion channels.
Patch clamping: a technique capable of measuring the currents flowing through single channels by Erwin Neher and Bert Sakmann at the Max Planck Institute in Germany in 1976 .
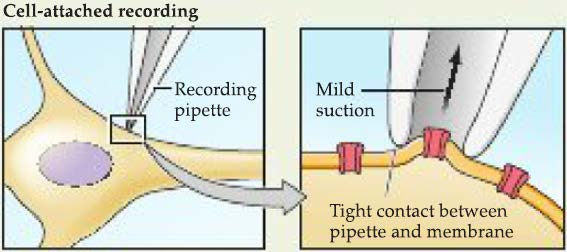
A glass pipette with a very small opening is used to make tight contact with a tiny area, or patch, of neuronal membrane.
All the current that flows when a single ion channel opens must flow into the pipette.
This minute electrical current can be measured with an ultrasensitive electronic amplifier connected to the pipette.
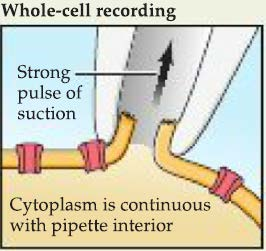
If the membrane patch within the pipette is disrupted by briefly applying strong suction, the interior of the pipette becomes continuous with the cell cytoplasm.
This arrangement allows measurements of electrical potentials and currents from the entire cell.
Patch clamping has allowed direct observation of microscopic ionic currents flowing through single ion channels.
Patch clamping confirms that voltage-sensitive Na+ and K+ channels are responsible for the macroscopic conductances(电导,电导系数) and currents (flowing through a large number of channels distributed over a much more extensive region of surface membrane) that underlie the action potential.
Functional studies of ion channels
To understand the functional significance of ion channel genes, the channels can be selectively expressed in well-defined experimental systems, such as cultured cells or frog oocytes, and then studied with patch clamping and other physiological techniques.
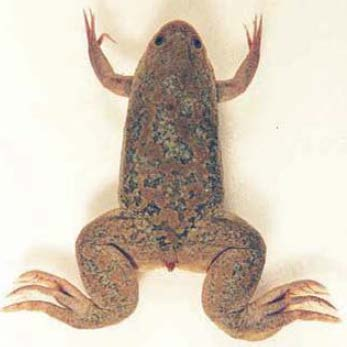
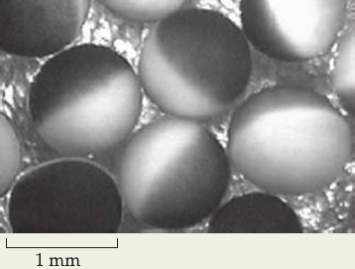
Oocytes are huge cells (approximately 1 mm in diameter) and are easily harvested from the female Xenopus.
In the early 1980s, Ricardo Miledi, Eric Barnard, and other neurobiologists demonstrated that Xenopus oocytes could express exogenous ion channels (after injecting mRNA), and that physiological methods could be used to study the ionic currents generated by the newly-synthesized channels.
Defined mutations (often affecting a single nucleotide) can be made in the part of the channel gene that encodes a structure of interest; the resulting channel proteins are then expressed in oocytes to assess the functional consequences of the mutation.
Channel genes can also be deleted from genetically tractable organisms, such as mice or fruit flies, to determine the roles these channels play in the intact organism.
Functional states of voltage-gated Na+ and K+ channels
Single channel studies show that the membrane of the squid axon contains at least two types of channels-one selectively permeable to Na+ and a second selectively permeable to K+. –ion selectivity.
Both channel types are voltage-gated, because their opening is influenced by membrane potential.
Both channel types must have a voltage sensor that detects the potential across the membrane.
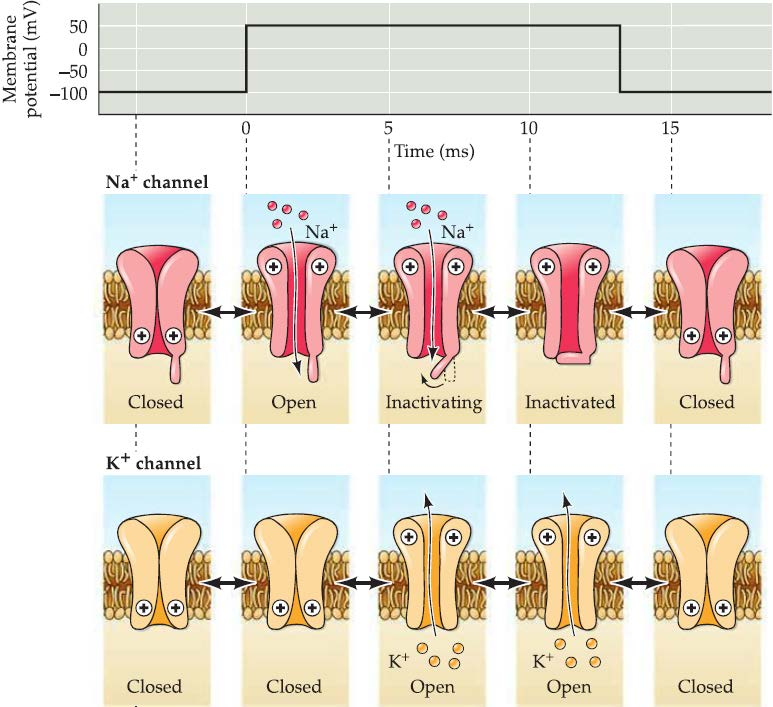
The gates of both channels are closed when the membrane potential is hyperpolarized.
When the potential is depolarized, voltage sensors (+) allow the channel gates to open–first the Na+ channels and then the K+ channels.
Na+ channels also inactivate during prolonged depolarization, whereas many types of K+channels do not.
These channel proteins provide unique binding sites for drugs and for various neurotoxins known to block specific subclasses of ion channels.
- Tetrodotoxin from puffer fish, saxitoxin from dinoflagellates, scorpion α- and β- toxins, batrachotoxin from frog, aconitine from buttercups, veratridine from lilies…
The diversity of ion channels
Over 100 ion channel genes have been discovered.
Ion channel genes contain a large number of coding regions that can be spliced together in different ways, giving rise to channel proteins that can have dramatically different functional properties.
RNAs encoding ion channels also can be edited, modifying their base composition after transcription from the gene.
Channel proteins can also undergo posttranslational modifications, such as phosphorylation by protein kinases, which can further regulate their functional characteristics.
Channels are often made up of subunits encoded by different genes, with different combinations of subunits producing channels of different function.
Although the basic electrical signals of the nervous system are relatively stereotyped, the proteins responsible for generating these signals are remarkably diverse, conferring specialized signaling properties to many of the neuronal cell types that populate the nervous system.
1. Voltage-gated ion channels
Voltage-gated ion channels are selectively permeable to each of the major physiological ions–Na+, K+, Ca2+, and Cl-.
Many different genes have been discovered for each type of voltage-gated channel.
- Na+ channel genes—SCN genes
- Ca2+ channel genes—CACNA genes
K+ channels
K+ channels are the largest and most diverse class of voltage-gated ion channels.
Nearly 100 K+ channel genes are known.
Different types of K+ channels were expressed in Xenopus oocytes, and the voltage clamp method was used to change the membrane potential and measure the resulting currents flowing through each type of channel.
They fall into several distinct groups that differ substantially in their activation, gating, and inactivation properties.
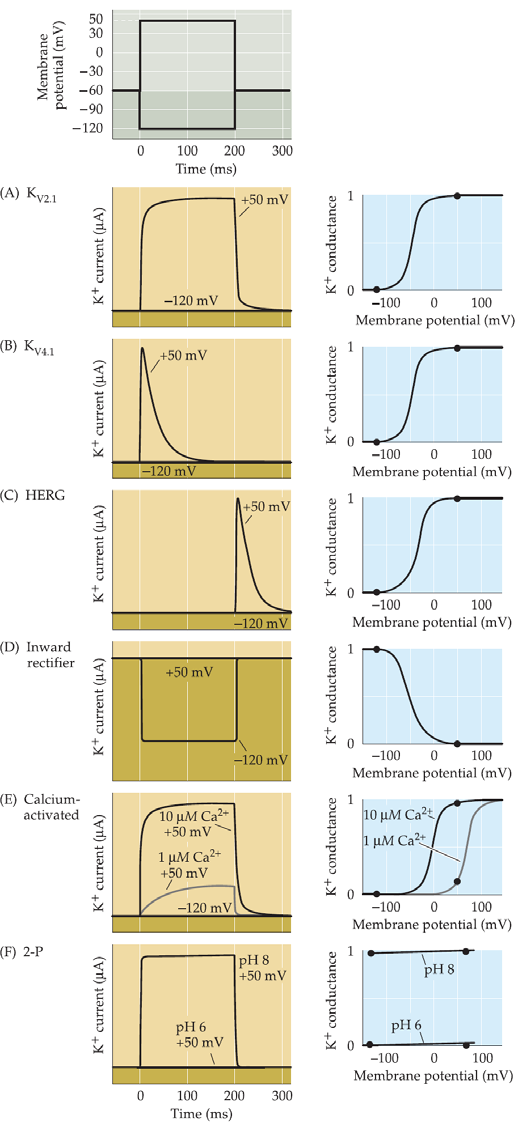
2. Ligand-gated ion channels
The most important ligand-gated ion channels in the nervous system is the class activated by binding neurotransmitters.
- These channels are essential for synaptic transmission and other forms of cell-cell signaling.
Other ligand-gated channels have their ligand-binding domains on their intracellular surfaces that interact with second messengers such as Ca2+, the cyclic nucleotides cAMP and cGMP, or protons.
- The main function of these channels is to convert intracellular chemical signals into electrical information.
Although many ligand-gated ion channels are located in the cell surface membrane, others are found in the membranes of intracellular organelles such as mitochondria or the endoplasmic reticulum.
3. Heat- and stretch-activated channels
Heat-activated ion channels such as some members of the transient receptor potential (TRP) gene family, contribute to the sensation of pain and temperature and help mediate inflammation.
Other ion channels respond to mechanical distortion of the plasma membrane and are the basis of stretch receptors and neuromuscular stretch reflexes.
The molecular structure of ion channels
Ion channels are all integral membrane proteins that span the plasma membrane repeatedly.
- Na+ and Ca2+ channel proteins consist of repeating motifs of six membrane-spanning regions that are repeated four times, for a total of 24 transmembrane regions.
- Accessory proteins, called β subunits, can regulate the function of these channels.
K+ channel proteins typically span the membrane six times, though there are some K+ channels that span the membrane only twice, or seven times, or four times.
Each of these proteins serves as a K+ channel subunit, with four subunits typically assembling to form a single functional ion channel.
- Chloride channels are structurally distinct from all other voltage-gated channels.
Most voltage-gated ion channels contain a distinct type of transmembrane helix that possesses a number of positively charged amino acids and serves as the voltage sensor that detects changes in the electrical potential across the membrane.
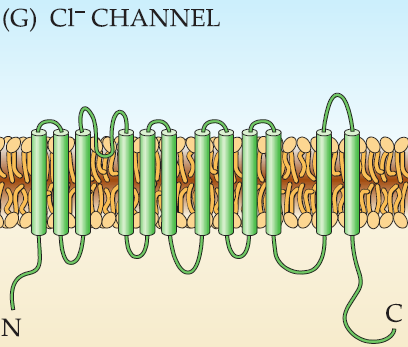
1. Structure of a simple bacterial K+ channel determined by crystallography
X-ray crystallography studies of K+ channels done by Rod MacKinnon at Rockefeller University. 2003 Nobel Prize.
Structure of one subunit of the channel, which consists of two helical membrane-spanning domains and a pore loop that inserts into the membrane.
Four subunits are assembled together to form a channel
In the center of the assembled channel, the channel pore can be observed as a narrow tunnel that allows K+ to flow through the protein and thus cross the membrane.
The channel pore is formed by the pore loops of each subunit, as well as by the membrane-spanning domains.
The structure of the pore is well suited for conducting K+; the narrowest part is near the outside mouth of the channel and is so constricted that only a nonhydrated K+ ion can fit through the bottleneck.
This part of the channel complex is responsible for the selective permeability to K+ and is therefore called the selectivity filter.
Deeper within the channel is a water-filled cavity that connects to the interior of the cell. This cavity evidently collects K+ from the cytoplasm and, utilizing negative charges from the protein, allows K+ ions to become dehydrated so they can enter the selectivity filter
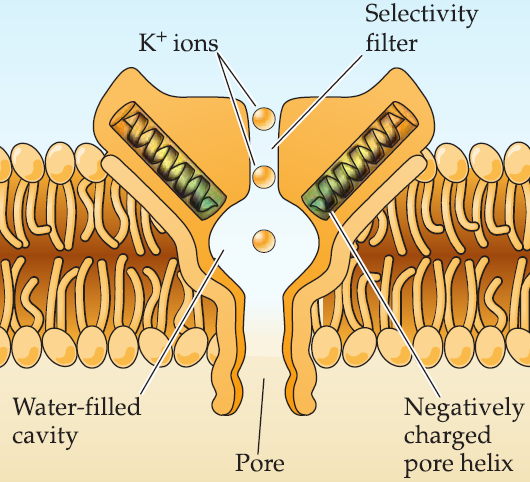
2. Structure of a mammalian voltage-gated K+ channel
Four subunits assemble to form the voltage-gated K+ channel.
The central pore region of this channel is very similar to that of the bacterial K+ channels, consisting of two membrane-spanning structures and pore loops contributed by each of the four subunits.
This voltage-gated channel has additional structures on its cytoplasmic side, such as a β subunit and a T1 domain that links the β subunit to the channel.
Most importantly, each channel subunit has four additional transmembrane structures that form the voltage sensors of this channel. These voltage sensors can be observed as separate domains that extend into the plasma membrane and are linked to the central pore of the channel.
The positive charges within these voltage sensors enable movement within the membrane in response to changes in membrane potential:
- One proposed model is that depolarization pushes the paddle-like sensors outward, while hyperpolarization pulls them inward.
- Such movements of the sensors then exert force on the helical linkers connecting the sensors to the channel pore, pulling the channel pore open or pushing it closed.
二、Transporters
Active transporters
Active transporters are plasma membrane proteins that generate and maintain concentration gradients for particular ions.
Active transporters form complexes with the ions they are translocating. The process of ion binding and unbinding for transport typically requires several milliseconds, which is much slower than ion movement through channels.
Active transporters gradually store energy in the form of ion concentration gradients, whereas the opening of ion channels rapidly dissipates this stored energy during relatively brief electrical signaling events.
Active transporters translocate ions against their electrochemical gradients, which requires the consumption of energy, and neuronal transporters fall into two classes based on their energy source:
1. ATPase pumps
ATPase pumps acquire energy directly from the hydrolysis of ATP
Na+/K+ ATPase pump is responsible for maintaining transmembrane concentration gradients for both Na+and K+.
Ca2+ pump provides one of the main mechanisms for removing Ca2+ from cells.
PMCA is found on the plasma membrane.
SERCA is used to store Ca2+ within the endoplasmic reticulum
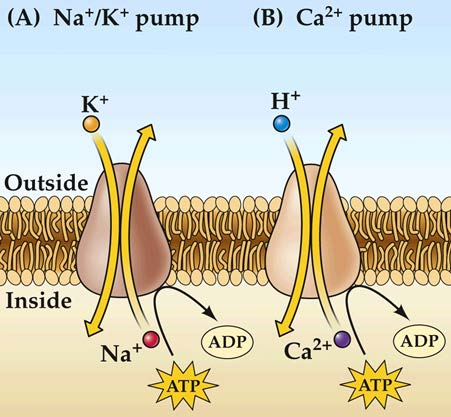
Molecular structure of ATPase pumps
Na+/K+ pump must exhibit several molecular properties:
- it must bind both Na+ and K+.
- it must possess sites that bind ATP and receive a phosphate group from this ATP.
- it must bind ouabain, the toxin that blocks this pump.
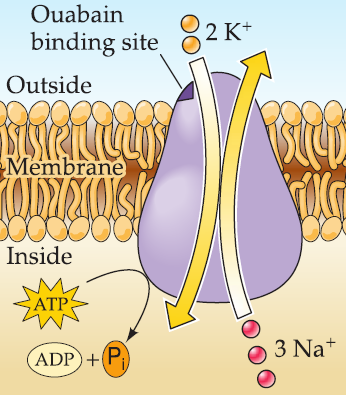
Na+/K+ pump is a large, integral membrane protein made up of at least two subunits, called α and β.
- the α subunit spans the membrane 10 times, with most of the molecule found on the cytoplasmic side.
- the β subunit spans the membrane only once and is predominantly extracellular.
- Functional domains.
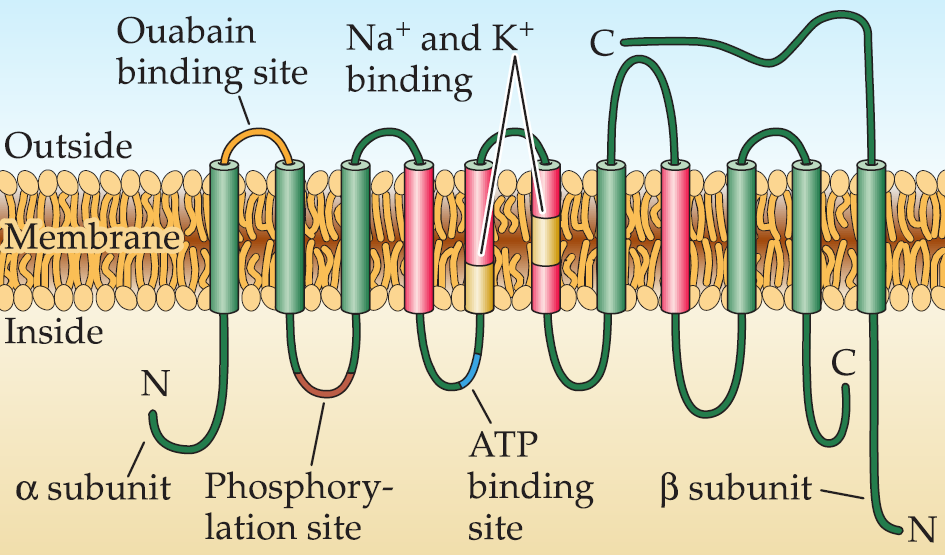
2. Electrochemical Gradients Used Active Transporter
A second class of active transporter does not use ATP directly but instead uses the electrochemical gradients of other ions as an energy source.
- Such transporters carry one or more ions up its electrochemical gradient while simultaneously taking another ion (most often Na+) down its gradient.
Ion Exchangers
ion exchangers exchange intracellular and extracellular ions.
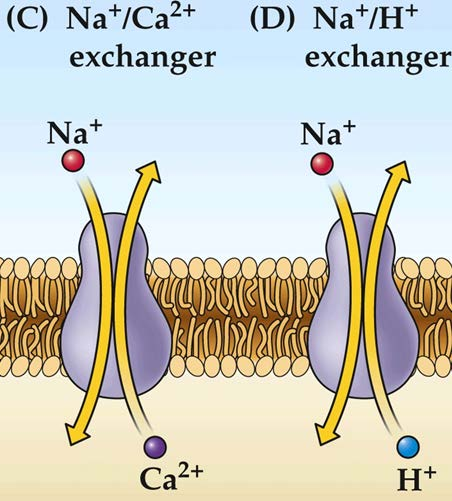
Na+/Ca2+ exchanger shares with the Ca2+ pump the important job of keeping intracellular Ca2+ concentrations low.
Na+/H+ exchanger, regulates intracellular pH.
Cotransporters
Cotransporters work by carrying multiple ions in the same direction.
Two such co-transporters regulate intracellular Cl- concentration by translocating Cl- along with extracellular Na+ and/or K+.
- Na+/K+/Cl- co-transporter transports Cl- along with Na+ and K+ into cells.
- K+/Cl- co-transporter swaps intracellular Cl- for extracellular K+
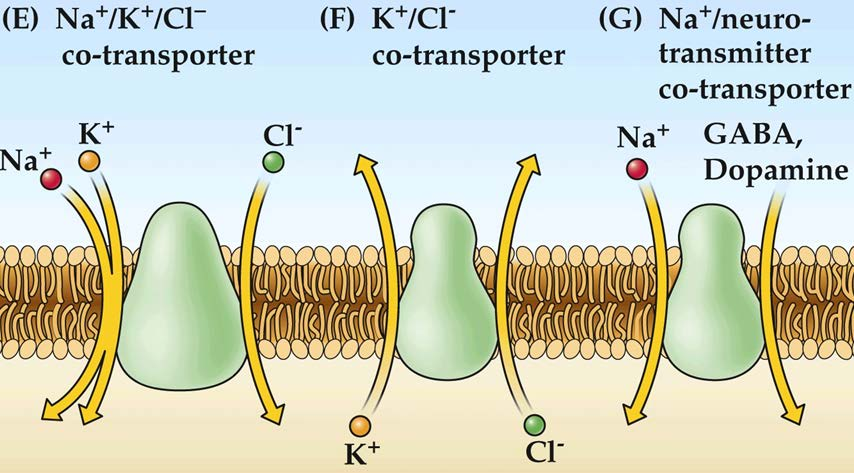
Na+/neurotransmitter co-transporters can transport neurotransmitters such as GABA and dopamine into synaptic terminals and glial cells.
Although the electrochemical gradient of Na+ (or other counter-ions) is the proximate source of energy for both ion exchangers and co-transporters, these gradients ultimately depend on the hydrolysis of ATP by ATPase pumps such as the Na+/K+ ATPase pump.
Functional properties of Na+/K+ pump
Best understood active transporter.
Its crucial importance for brain function is evident from the fact that this transporter accounts for 20%-40% of the brain’s total energy consumption.
The Na+ pump was first discovered in neurons in the 1950s, when Richard Keynes at Cambridge University used radioactive Na+ to demonstrate the energy-dependent efflux of Na+ from squid giant axons
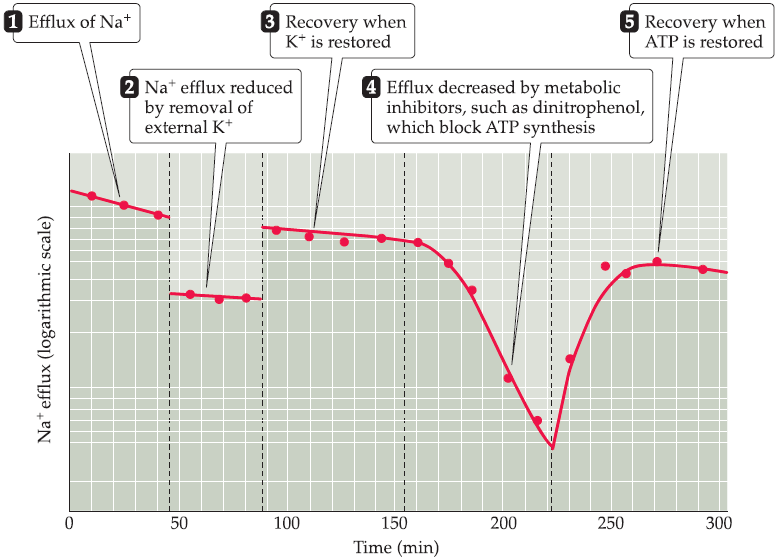
These energy-dependent movements of Na+ and K+implicated an ATP-hydrolyzing Na+/K+ pump in the generation of the transmembrane gradients of both Na+ and K+.
Uphill movements of Na+ and K+ are driven by ATP, which phosphorylates the pump.
Phosphorylation switches the pump from exporting Na+ to importing K+.
These ion fluxes are asymmetrical, with three Na+carried out for every two K+brought in.
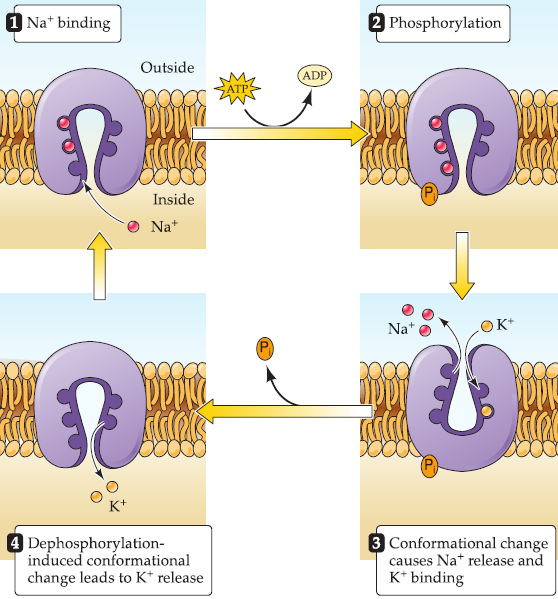